In 1797 Henry Cavendish, one of Great Britain’s leading scientists, built a contraption to weigh the world.
At the time, Earth’s mass was unknown, as was its composition. Was it mostly solid rock? Did it vary with depth? Astronomer Edmond Halley even suggested that Earth might be hollow. Isaac Newton had compared Earth’s mass with that of other bodies in the solar system and knew, for example, that Earth was more massive than the moon. He had even suggested a way to determine Earth’s absolute mass: measure the gravitational attraction between two small spherical masses with great accuracy, then extrapolate Earth’s own mass from the result. But Newton summarily dismissed his own idea—he thought the attraction between the spheres would be too small to detect, even with impractically large masses. “Nay, whole mountains will not be sufficient to produce any sensible effect,” he wrote in his masterpiece, the Principia, which laid out his laws of motion and gravitation.
On an August day more than a century later Cavendish proved Newton wrong. The device he had built in a shed on his estate in southwest London consisted of two 1.6-pound lead balls attached to opposite ends of a six-foot-long wood rod, which hung from a wire fastened to an overhead beam. Two much heavier lead spheres, each weighing nearly 350 pounds, were suspended separately about nine inches away from the lighter balls. Cavendish expected that the gravitational pull of the heavy spheres on the smaller ones would make the wood rod rotate ever so slightly, and he was right—it moved just over a tenth of an inch.
On supporting science journalism
If you're enjoying this article, consider supporting our award-winning journalism by subscribing. By purchasing a subscription you are helping to ensure the future of impactful stories about the discoveries and ideas shaping our world today.
This allowed him to directly measure the gravitational force exerted by each of the larger spheres on the smaller ones. Because he already knew that Earth exerted a gravitational force of 1.6 pounds on each of the small spheres (in the English system of units, a pound is by definition a measure of force), Cavendish could set up a simple ratio: the gravitational force between the small sphere and the large sphere compared with the gravitational force between the small sphere and Earth. Because the gravitational force is directly proportional to the masses being measured, he could use that ratio to solve for Earth’s unknown mass. Over the course of nine months he repeated the experiment 17 times and found that Earth weighed 13 million billion billion pounds, a result essentially identical to the best modern estimates.
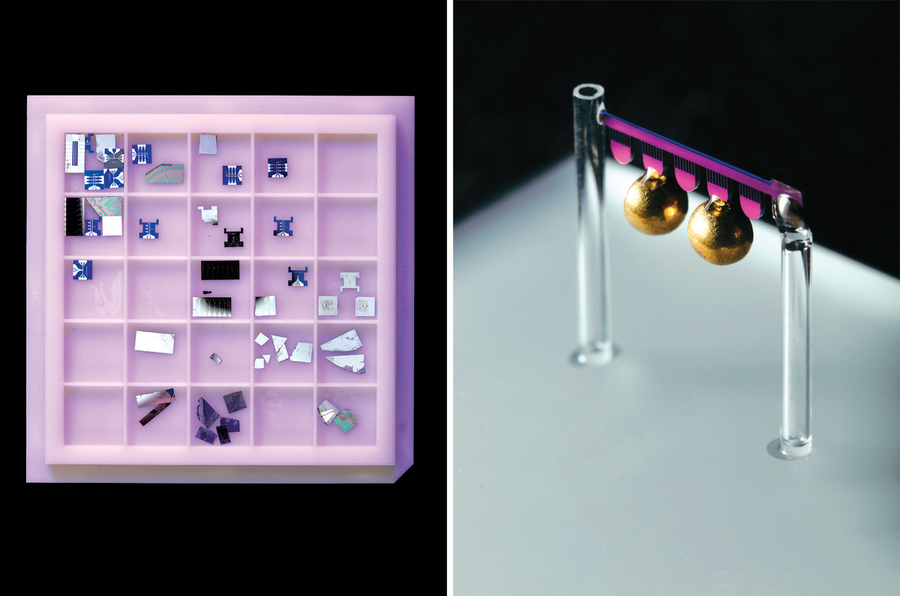
Superconducting circuits (left) aid the levitation experiment. Researchers are also trying to measure the gravitational fields of millimeter-wide gold spheres (right) to observe gravity closer to the quantum realm. Credit: Mattia Balsamini
“It’s an incredible story,” says Markus Aspelmeyer, who has been recounting the Cavendish experiment during a Skype call. “It was the first precision tabletop experiment [with gravity].” Cavendish’s 220-year-old tour de force, though not actually conducted on a tabletop, is a source of inspiration for Aspelmeyer, a physicist at the University of Vienna in Austria. Like Cavendish, he has plans for an ambitious, seemingly impossible experiment, one that might transform our understanding of gravity: he wants to use a small-scale setup—literally on a tabletop in his lab—to find evidence that gravity might be a quantum phenomenon.
Of the four fundamental forces in the universe, gravity is the only one that cannot be described by the laws of quantum mechanics, the theory that applies to all other forces and particles known to physics. Electromagnetism; the “strong” nuclear force that binds atomic nuclei; and the “weak” nuclear force that causes radioactive decay—they are all quantum to the core, leaving gravity as a sole, mysterious outlier.
This exception has vexed physicists since Albert Einstein’s heyday. Einstein never managed to unify his own theory of gravity—the general theory of relativity—with quantum mechanics. Most physicists who now work on the problem believe that the unification occurs when we zoom in on the cosmos to what is called the Planck scale, after Max Planck, one of the founders of quantum theory. Distances on the Planck scale are so tiny—100 trillion trillion times as small as a hydrogen atom—that spacetime itself is thought to assume quantum characteristics. A quantum spacetime would no longer be the smooth continuum described by general relativity; it would be coarse-grained, like a digital photograph that becomes pixelated when magnified. That graininess is a hallmark of quantum theory, which confines the energy, momentum and other properties of particles to discrete bits, or quanta. But what exactly is a quantum of spacetime? How could time or distance be measured if space and time themselves are fractured like broken rulers?
“All our theories of physics either explicitly or implicitly require the existence of rods and clocks: something occurred [here] at this time and then did this [there] at a later time,” says Miles Blencowe, a theoretical physicist at Dartmouth College. “Where do you start if you don’t even have a time parameter or a distance parameter?” Lajos Diósi, a theoretical physicist at the Wigner Research Center for Physics in Budapest, sums up the conundrum this way: “We don’t know what will be there, but we know for sure that there will be a total scrambling of the spacetime continuity if you go down to the Planck scale.”
Unfortunately for physicists, there is no way to observe phenomena on the Planck scale and thus no way to check the predictions of various theories of quantum gravity to see which of them might be right. “The situation is not that we do not have theories of quantum gravity,” says Carlo Rovelli, a theoretical physicist at Aix-Marseille University in France. “We do. The problem is that we have more than one.”
In physics, the higher the energy scale of your experiment, the smaller the distance you can probe. And probing the Planck scale directly would require a machine more than 15 orders of magnitude more powerful than CERN’s Large Hadron Collider (LHC) near Geneva, the largest particle accelerator ever built, with a circumference of 27 kilometers. As one physicist says, such an accelerator would need to be roughly the size of our galaxy. Machines such as the LHC bash particles together at nearly the speed of light, and physicists hope something new will emerge from the debris. The basic approach is not much different from blowing up a safe to find out what is inside. The practitioners of tabletop physics aim to replace brute force with finesse, like safecrackers listening to the tumblers of a lock clicking into place. “You’re trading high energy for high precision is the way I look at it,” says Eric Adelberger, a physicist at the University of Washington. “There’s the energy frontier, and there’s the precision frontier. If you can measure something really, really well, you can test physics that’s going on at some really high-energy scale.” Now at least three groups, including Aspelmeyer’s, are designing experiments to do just that. The scientists are optimistic that these projects will finally reach the levels of precision needed to probe the realm where gravity goes quantum.
A Thought Experiment
To understand why precision allows physicists to indirectly access higher energies and thus smaller scales, consider a historical analogue: Brownian motion. In a paper published in 1905, Einstein showed that the puzzling random movements of pollen grains in a jar of water could be explained by collisions with water molecules, even though the molecules themselves were many orders of magnitude too small to be observed directly. Aspelmeyer and other physicists are betting that the unobservably small things happening in the Planckian realm might similarly influence phenomena accessible to tabletop experiments. And although particle accelerators cannot be upgraded by orders of magnitude—we are unlikely to see accelerators with 1,000-kilometer circumferences—the precision of tabletop experiments may well improve by a few orders of magnitude in the decades ahead.
Such gains might allow Aspelmeyer to test a key assumption shared by all theories of quantum gravity: that gravity itself should display some profoundly strange quantum properties. “If that is really true, there should be some consequences for phenomena at an energy scale that is much, much smaller [than the high energies that correspond with the Planck scale]”—that is, at roughly the scale we inhabit, Aspelmeyer says. “The question is: Can we come up with experiments that possibly test those consequences?”
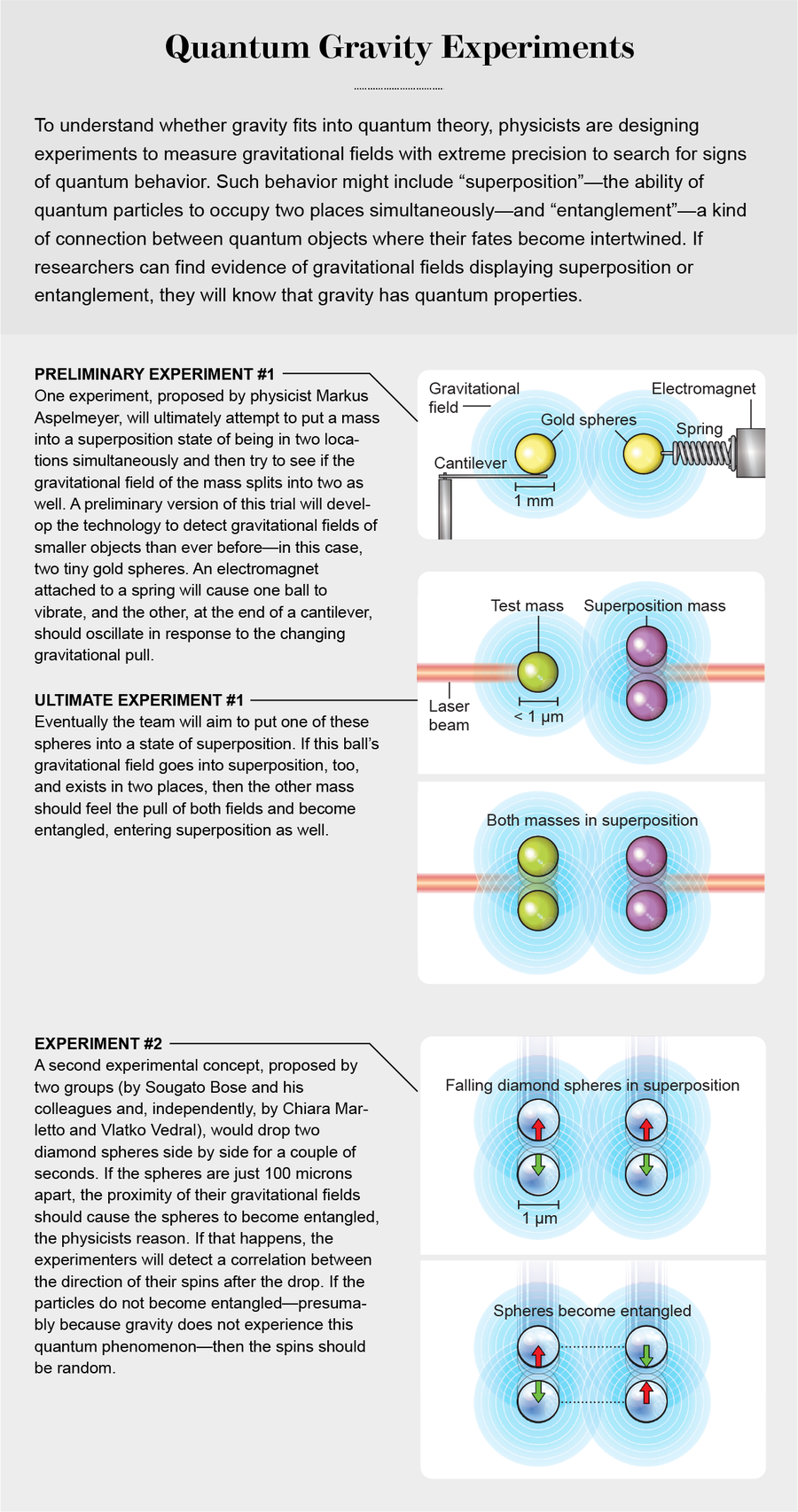
Credit: Ben Gilliland
What Aspelmeyer has in mind is an experiment that would measure the gravitational attraction between two spherical masses. Unlike Cavendish, though, Aspelmeyer will not be weighing Earth, and his milligram masses are orders of magnitude smaller than Cavendish’s lead balls. He wants to test whether gravity interacts at all with the quantum properties of small masses. Specifically, he intends to look at what kind of gravitational effects might be generated by an object placed in a “Schrödinger’s cat”–like state of being both here and there at once.
In the quantum world, particles have the uncanny ability to be in two places simultaneously—a superposition, as physicists call it. Scientists have observed quantum superpositions many times in laboratories, but they are delicate states. Interactions with any nearby particles quickly cause objects in superposition to “collapse” into a single position. But Aspelmeyer wonders what properties these particles have while the superpositions last. Do they create their own minuscule gravitational fields, for instance? “Imagine you place an object in a superposition,” he says, “and now you ask a question: How does it gravitate? That is the question we want to answer.”
The experiment Aspelmeyer hopes to carry out was first proposed as a gedankenexperiment—a thought experiment—by the legendary physicist Richard Feynman at a conference in 1957. Feynman argued that if gravity is indeed a quantum phenomenon, a superposition of a particle in two places at once will create two separate gravitational fields. According to the general theory of relativity, gravitational fields are distortions of space and time. Thus, in the case of a small mass in a quantum superposition, two different spacetimes would coexist side by side, almost like two separate mini universes, a state of affairs that should not exist in Einstein’s theory.
If that spacetime superposition arose, how would another object—a test mass—interact with it? Would the motion of the test mass indicate that it had felt the pull of two different gravitational fields? Or would the interaction cause the superposition to collapse, as some physicists believe, resulting in normal gravitational dynamics? If the superposition persisted and if the test mass did interact with the superposition’s gravitational fields, it would be strong evidence that the test mass and the superposition had become “entangled”—a telltale feature of quantum mechanics where the properties of two separate particles become inextricably linked. Feynman argued that because only quantum phenomena can become entangled, the experiment would show that gravity, like all other known forces in the universe, is fundamentally quantum.
Such an outcome would not in itself validate any particular theory of quantum gravity, but it would be indirect evidence that gravity is quantized on the Planck scale. Even more broadly, the experiment would provide compelling evidence that the laws of quantum mechanics hold at all scales, not just in the realm of photons, atoms and other fundamental particles. Some physicists have clung to the idea that quantum mechanics might break down when it comes to describing the macroscopic world. Roger Penrose, for example, a physicist at the University of Oxford, and Diósi have suggested that gravity causes superpositions above a certain size to collapse, effectively dividing the quantum world from the so-called classical one.
“One of the areas where quantum theory is supposed to fail is when it comes to describing gravity,” says Chiara Marletto, a theoretical physicist at Oxford. “There have been a number of eminent scientists who maintain that gravity will be exactly the place where quantum theory breaks down. So, instead of having a quantized [theory of] gravity, we should actually make quantum theory classical for it to describe gravity.” In this way of thinking, quantum theory might need to be modified to make it consistent with general relativity, rather than trying to fit gravity into quantum theory as it is.
Turning Thought into Reality
The technology and expertise needed to decide the issue did not exist when Feynman came up with his idea, and even now the project remains daunting. For several years Aspelmeyer’s lab has been pushing to measure the gravitational fields of ever smaller masses. It is a tricky undertaking: Earth’s enormous gravity swamps the fields of even relatively large objects. The smallest mass for which a gravitational field has been measured so far is a 700-milligram tungsten sphere. That is about the mass of a paper clip or a raisin—a gargantuan object compared to quantum particles.
To realize Feynman’s thought experiment, Aspelmeyer and his colleagues will need to work with objects considerably smaller than paper clips. They are now developing a prototype experiment to detect the gravitational fields of millimeter-wide gold spheres (gold was chosen for its density and purity) weighing just a few tens of milligrams. “That’s a factor of tens or hundreds less heavy than anything else that has been measured so far,” Aspelmeyer says. In the experiment, the researchers will place two gold spheres a few millimeters apart, with one attached to a small, spring-mounted magnet and the other fixed to the end of a micromechanical cantilever. When the electromagnet is turned on, the sphere on the spring will start vibrating, creating a changing gravitational field that in turn makes the mass on the cantilever bounce up and down like a diver on a board. The cantilever’s motion—tracked by lasers—essentially amplifies the gravitational force of the sphere attached to the spring, making it easier to detect against the background of Earth’s field.
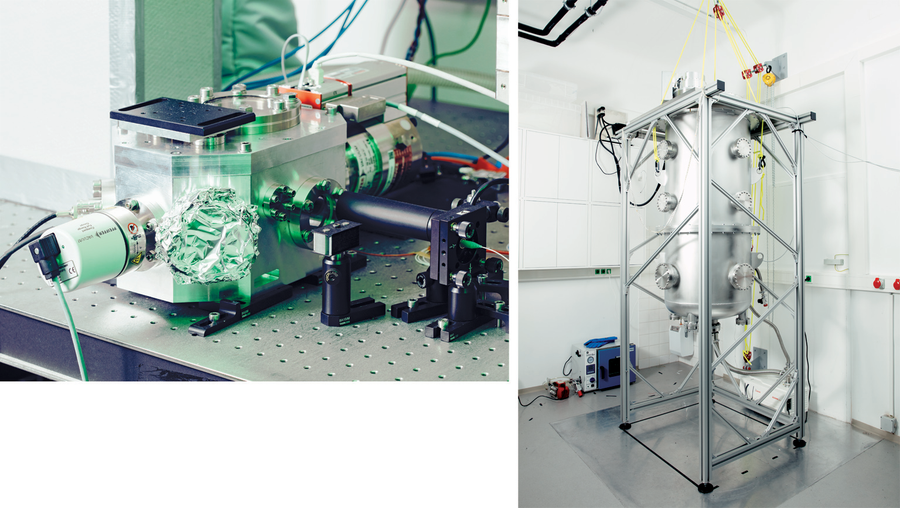
Vacuum chambers isolate small masses from the outside world to measure their gravitational fields with minute precision. Credit: Mattia Balsamini
After honing their gravitational-measuring skills with ordinary, nonquantum masses, Aspelmeyer’s team would then tackle superpositions. If he could put two small spheres into superpositions, Aspelmeyer could test how their gravitational fields interacted. The results could suggest that the particles were entangled, supporting Feynman’s intuition about gravity’s quantum nature.
What will it take to pull all this off? To have a realistic shot at creating a quantum superposition, Aspelmeyer will need to shrink his millimeter-size gravitational test masses down to fractions of a micron—a 1,000-fold reduction. At the same time, he will need superpositions of objects that are massive enough to have detectable gravitational fields. For that he will likely draw on the talents of a colleague at Vienna, Markus Arndt, who holds the record for the largest object ever placed in a superposition: a behemoth of a molecule containing more than 800 atoms. And instead of being stuck to springs and cantilevers, the masses would be suspended in space with “optical tweezers”—tightly focused laser beams.
“If I can detect the gravitational field of an object over which I can obtain quantum control, then I am in business,” Aspelmeyer says. “This would be the long-term dream—not tomorrow, not in five years. Both from the top down and bottom up—from making [the gravitational] masses smaller and making the [superposition] masses larger—we think we know how to get there and bring those two domains together. Now we just need to work hard.”
Arndt, Aspelmeyer’s likely collaborator, says the experiment presents a host of challenges: the small, spherical masses will be difficult to isolate gravitationally and prone to interacting with any nearby surface. “There are so many effects that are hard to suppress,” he says. “Still, it has to be tried, by all means. If we don’t start now, it won’t be done in 10 years.” Arndt compares the effort that will be required with the search for gravitational waves, a phenomenon predicted by Einstein’s general theory of relativity. In 2015 the giant Laser Interferometer Gravitational-wave Observatory (LIGO) finally detected the first gravitational wave, but the discovery was a long time coming. “It was a 40-year effort to get the gravitational-wave detector going,” Arndt says.
The Last Refuge of Quantum Holdouts
Aspelmeyer is not the only physicist working on the problem. In December 2017 two independent groups simultaneously published their own very similar takes on Feynman’s thought experiment. Sougato Bose, a physicist at University College London, and his colleagues and Marletto and her Oxford colleague Vlatko Vedral described a way to test for the gravitational entanglement between superpositions of microscopic particles without having to measure their gravitational fields.
In the proposed experiment, pairs of micron-wide diamond spheres would be put into superpositions and allowed to fall in a vacuum for a couple of seconds in Earth’s gravitational field. If the spheres were close enough together—about 100 microns apart, according to Bose’s estimates—their gravitational fields should cause the particles to become entangled. When that happens, the properties of the entangled particles will instantaneously correlate in ways that are not possible in classical physics. One particle’s spin, for example—whether it points up or down in a magnetic field—will flip in the opposite direction as soon as the spin of its entangled partner is measured.
By tracking how often such correlations occur—Bose says that 10,000 trials should yield an answer—he, Marletto and Vedral could determine whether the falling diamonds had indeed become entangled. Once again, entanglement would suggest that gravity itself must have quantum properties. “Our work will prove that gravity is quantum in the sense that it obeys the superposition principle,” Bose says. The experiment faces many of the same challenges that Aspelmeyer’s does: it needs large superpositions that last for seconds at a time and stay close enough together so that gravity can entangle them. “That makes the thing very difficult,” Bose says. “But I’m sure I’ll see it in my lifetime.”
Both experiments, if they pan out, would give physicists their first indirect evidence that gravity—and therefore spacetime itself—must be quantized on the Planck scale. And that is an exciting prospect for Rovelli and other quantum gravity theorists, who have spent years working on theories without any experimental feedback. “I think it’s a game changer, this idea, the attempt to see quantum gravity in the lab,” Rovelli says. “As far as we know [gravity’s quantum nature] should definitely be real; otherwise we haven’t learned a thing about the world.”
A century after its birth quantum mechanics remains the most baffling of scientific theories. Some physicists, most famously Einstein, doubted that it could be the final word on the nature of reality. Yet countless experiments have confirmed the theory’s predictions, typically with multidecimal-place accuracy. In some sense, the question of whether gravity is quantum or classical represents a last refuge for those who feel that there must be something wrong with quantum mechanics. If these tabletop experiments succeed, that refuge will crumble.
“Quantum theory teaches us a completely different way of describing what we can say about nature,” Aspelmeyer says. “The rule book that we have found through quantum theory is a fundamental one and has to apply in general to all the theories we have.”