I was walking out of a classroom at the Massachusetts Institute of Technology, where students and I had been talking about the way planets form, when I was stopped by my colleague Ben Weiss. He studies magnetism in space rocks, and he was very excited. Weiss pulled me down the hall to his office to show me new data on one of these rocks, a meteorite called Allende. It was information that could change almost everything planetary geologists thought about the solar system. It was 2009, and that fall Weiss's research team had shown that Allende—which crashed into Earth in a huge fireball in Mexico in 1969 and contained some of the oldest known material in our system—harbored signs of an ancient magnetic field in its rock. The discovery was a surprise. This kind of field, astronomers thought, was made only by a magnetic dynamo of intensely hot, flowing liquid metal inside a planet, the way Earth's magnetic field is produced by liquid iron spinning in the planet's core. But Allende was supposed to be a fragment from a parent planetesimal—an early, nascent planet—that had been only slightly warm. Scientists presumed it had never gotten hot enough to melt the metal it contained. How, then, Weiss wondered, could this ancient piece of our solar system have become hot enough to create a magnetic dynamo?
My students had just been pelting me with questions about planetary evolution, challenging me to rethink some textbook wisdom, so I happened to have the bare bones of a new idea that might help answer Weiss's question. I went over to his whiteboard and began sketching it out.
Planetesimals had long been known to contain short-lived, unstable aluminum atoms radiating excess nuclear energy. This radioactive isotope is called 26Al, and when it decayed, that excess energy could have heated planetesimals. Conceivably, the heat from 26Al in Allende's parent body could have climbed so high that the object actually melted from the inside out. Metal within the body would have separated from silicate minerals in the rest of the rock and formed a liquid core that started to spin as the space rock rotated, creating a magnetic dynamo. Meanwhile the outside of the planetesimal would have been chilled by the cold of space, and cold rock and dust from our solar system's primitive disk would have kept adding to this unmelted rind.
On supporting science journalism
If you're enjoying this article, consider supporting our award-winning journalism by subscribing. By purchasing a subscription you are helping to ensure the future of impactful stories about the discoveries and ideas shaping our world today.
This idea that early building blocks of the solar system contained so much energy was not the story I had learned in high school. Textbooks often still say that the solar system formed in a quiet, stately manner. That creation, 4.567 billion years ago, was thought to be an orderly, civilized process, like a minuet: the gas and dust of a molecular cloud spun down into a disk around a growing young star, and the gas and dust coalesced into many little boulders, each of which gradually grew to tens to hundreds of kilometers in diameter. These planetesimals themselves then rammed together to form larger bodies, each perhaps the size of Mars, called planetary embryos. Only after that did the temperature in this space nursery pick up. These embryos, which in growing had gained gravity strong enough to begin clearing their orbits of surrounding debris, then collided and grew into planets. Eventually components in these planets separated into the familiar churning metal core and a silicate mantle—hot, wildly volcanic places, antithetical to life.
.png?w=900)
That's the old view. By the time Weiss and I began pondering Allende, some other data, too, indicated that the earliest solar system was really a place of rapid and violent change. Now that tame sequence of dust to boulders to planetesimals to embryos to planets is being replaced. Actual planetesimal formation, once surmised to take place over hundreds of millions of years, happened in just about three million years. If the age of our solar system, in human time, is now a day, this growing up happened during the very first minute. More energy in the early small components—energy like that provided by the aluminum heating and early collisions—means they did not have to wait and grow before developing different layers. Relatively minute planetesimals could host processes previously believed to be confined to planets, from melting and degassing to the creation of magnetic dynamos and volcanism.
And things in this system did not simply grow from small to large. Often large things blew apart into smaller pieces. Planet-size spheres may have formed in those early years through collisions of these smaller high-energy bodies, but glancing, hit-and-run blows among planetesimals sometimes stripped or destroyed them instead. Their debris could hit other spheres, enlarging them to planet size. Planets could be built, torn asunder and rebuilt in just 10 million years or less.
The Disappearing Disk
Planetary scientists pieced together this novel, three-ring-circus vision of the active young solar system with great help from new tools for calculating the ages of meteorites and of planetary dust clouds—similar to our primordial solar system—elsewhere in the cosmos.
Over the past 15 to 20 years scientists have developed instruments capable of measuring the elements that make up space rocks to parts per million or even less. Because we have a good idea of how long it takes radioactive elements to decay into their daughter elements, such measurements allow us to date when the planets and planetesimals that shed these fragments formed and changed. Scientists all over the world—notably Alex Halliday of Columbia University; Thorsten Kleine of the University of Münster in Germany; Stein Jacobsen of Harvard University; Mary Horan and Rick Carlson, both at the Carnegie Institution for Science; and Richard Walker of the University of Maryland—have measured collections of meteorites. The work made clear that planetesimals formed within the first few million years after the dusty disk began to cool, that many of our terrestrial planets could have formed within the first 10 million years, and that even most of Earth probably formed and differentiated into a core and mantle by a few tens of millions of years.
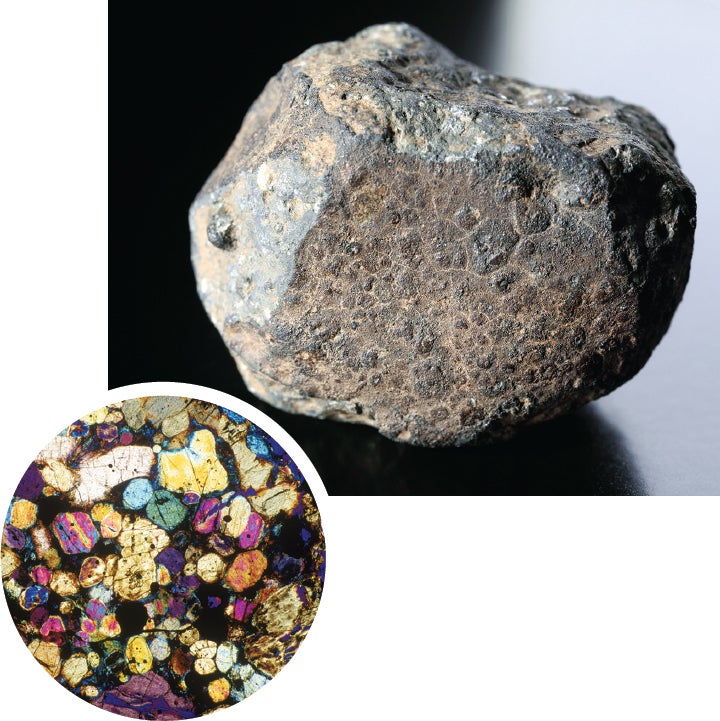
Ancient magnet: a fragment of a meteorite called Allende (shown in photograph and micrograph cross section) holds signs of magnetism created by planet precursors. Credit: Detlev Van Ravenswaay Science Source (photograph); Michael AbbeyScience Source (micrograph cross section)
Other avenues of research have yielded similar results. With ever improving telescopes, we can see young stars growing in other parts of the Milky Way, and in some cases, we can see the dust and gas disk from which a star and its planets grow. By estimating the ages of stars orbited by planets and comparing those measures with estimates for stars surrounded only by disks of dust and gas, researchers determined about 15 years ago that these disks last, on average, only three million years.
Planetesimals, therefore, have just three million years to grow on average. Any dust and gas not accreted onto rocks by then is lost, burned in the star or dispersed in space, and no more material for planet building is available. Considering that theorists used to think accretion took hundreds of millions of years, this is quite an acceleration!
More evidence for this timing comes from using the decay of radioactive elements like a clock that ticks away at a steady rate as one element turns into another. The new instruments gave teams throughout Europe and the U.S. enough precision to measure these elements and thereby learn how long that clock had been ticking. Meteorites that have fallen to Earth contain those elements. Most are pieces of asteroids, which themselves are primitive remnants of planetesimals. (Some other meteorites are from the moon, some are from Mars and others are from bodies not yet identified.)
One radioactive isotope of the element hafnium prefers to stay in silicate minerals, such as those in Earth's mantle. But it decays to an isotope of tungsten, which combines readily with metals that make up planet cores. This decay happens on a regular schedule: half the hafnium turns into tungsten in nine million years. This system gives us the timescale of metal-silicate (core-mantle) differentiation of planets and planetesimals: metal core formation scavenges tungsten from the silicate mantle and carries it into the growing core. Any hafnium stays happily in the mantle and keeps on decaying into tungsten, which then remains in the silicate mantle if no further core formation occurs. When scientists measure the ratios of hafnium to tungsten in meteorites, the amount of that tungsten isotope in them gives the time since core formation.
Such isotopic measurements of iron meteorites—many of which presumably hail from the metallic cores of planetesimals—show that their parent bodies formed within just 500,000 years of the first solids condensing from our protoplanetary disk of dust and gas. That time is less than 10 seconds into our putative 24-hour solar system. If iron meteorites are core fragments of planetesimals smashed apart by impacts, then planetesimals must have actually formed, melted and created iron cores within that tiny period.
Growth Spurt
With experimental data firmly showing that the solar system formed much more quickly than textbook scenarios indicated, scientists who study it had to explain how planets came into being so quickly. That put the ball in the theorists' court. How can dust and pebbles just microns to centimeters in diameter, all orbiting around our young sun, clump together to form bodies up to 10 million times larger (100-kilometer planetesimals) in just 500,000 years?
The answer is not obvious. Standard physics says small clumps of dust that collide can readily stick together through electromagnetic forces, much the way static electricity creates clumps of household dust. The energy absorbed as a result of compression and loss of porosity during collisions also helps clumps stick together rather than bouncing off one another or breaking apart. As the clumps grow, though, they reach what is called the meter barrier. Before they reach a meter in diameter, these growing boulders become too large to stick by electromagnetic forces while still being too small to stick through gravitational attraction. Impacts even at very low speeds cause these conglomerates to destruct rather than accrete. Yet we know that such materials must be able to grow from meter size to planetesimal size—the planet we stand on today is a testament to that. So some other processes must have been at work.
Several ideas for how growth beyond the meter barrier occurs have been proposed. Most hypotheses involve concentrating material in the protoplanetary disk through various types of turbulence that slam particles together. Such whirlpool-like forces might include phenomena called Kelvin-Helmholtz eddies, which develop between the gas and dust layer of the disk and could effectively crush regions of material together into larger bodies. Much of that work has been pioneered by Anders Johansen, now at Lund University in Sweden. Hal Levison of the Southwest Research Institute and Johansen have separately worked on another model, called pebble accretion. His calculations indicate that even the smallest pieces of dust and clumps can be gravitationally diverted over several orbits to add to a growing planetesimal and can do so fast enough to build planetesimals early in the life of the solar system.
Melting in a Freezer
No kind of crushing, however, could have caused the planetesimals to differentiate into mantles and cores. If planetesimals were first formed of primordial disk material in which metals and silicates were intimately mixed, then only high temperatures and at least partial internal melting would allow the metal to sink to the interior and form a core. Calculations show that slamming these relatively small bodies together would not provide enough energy to melt them. Consequently, researchers were left wondering where that melting energy—within the vast freezer of chilly space—came from.
This is where ideas about radioactive aluminum come in. Each time one of these atoms decays, it gives off a burst of heat. These tiny quanta of heat could have added up to a powerful energy source in the early solar system. Because aluminum is one of the six most common elements in stony material (the others are silicon, magnesium, iron, oxygen and calcium), 26Al, which has a half-life of about 700,000 years, could easily have heated at least some planetesimals to a melting temperature.
But what would have kept them from melting completely, considering that the recent observations strongly indicate that some of these planetary seeds had unmelted rinds on the outside? Size is part of the answer. In the case of Allende's parent planetesimal, to reach melting, the rocky body would have needed to be massive enough so that its interior produced heat faster than its exterior radiated it away. A larger body can get hotter inside than a smaller body can because it has a greater heat-producing volume compared with its heat-releasing surface. But the short half-life of 26Al means this growth had to be quick. To retain enough heat for the melting pattern we envisioned, Allende's parent planetesimal would have grown to 10 kilometers or more in radius within about two million years of the first solids in the solar system (the equivalent of the first 37 seconds of our 24-hour solar system)—and we think it may have grown to as much as 200 kilometers in radius.
It had been thought that planetesimals either melted entirely or remained primitive. But Weiss and I were suggesting a hybrid, where the most primitive material in the solar system encased a planetesimal that had melted inside—an outer rind as well as a melted core. This made sense because the Allende meteorite—with its record of a magnetic field caused by a heated interior—consists of unheated, primitive material alone. The only place it could come from would be a cool outer rind. Allende's parent planetesimal retained this primitive unmelted surface rind because it was chilled by the coldness of space and because dust in the cool protoplanetary disk continued to attach to it over time. Unchanged by heating, the rind was able to maintain a record of the magnetic field produced by the inner part of the planetesimal's structure, the melted core and its magnetic dynamo.
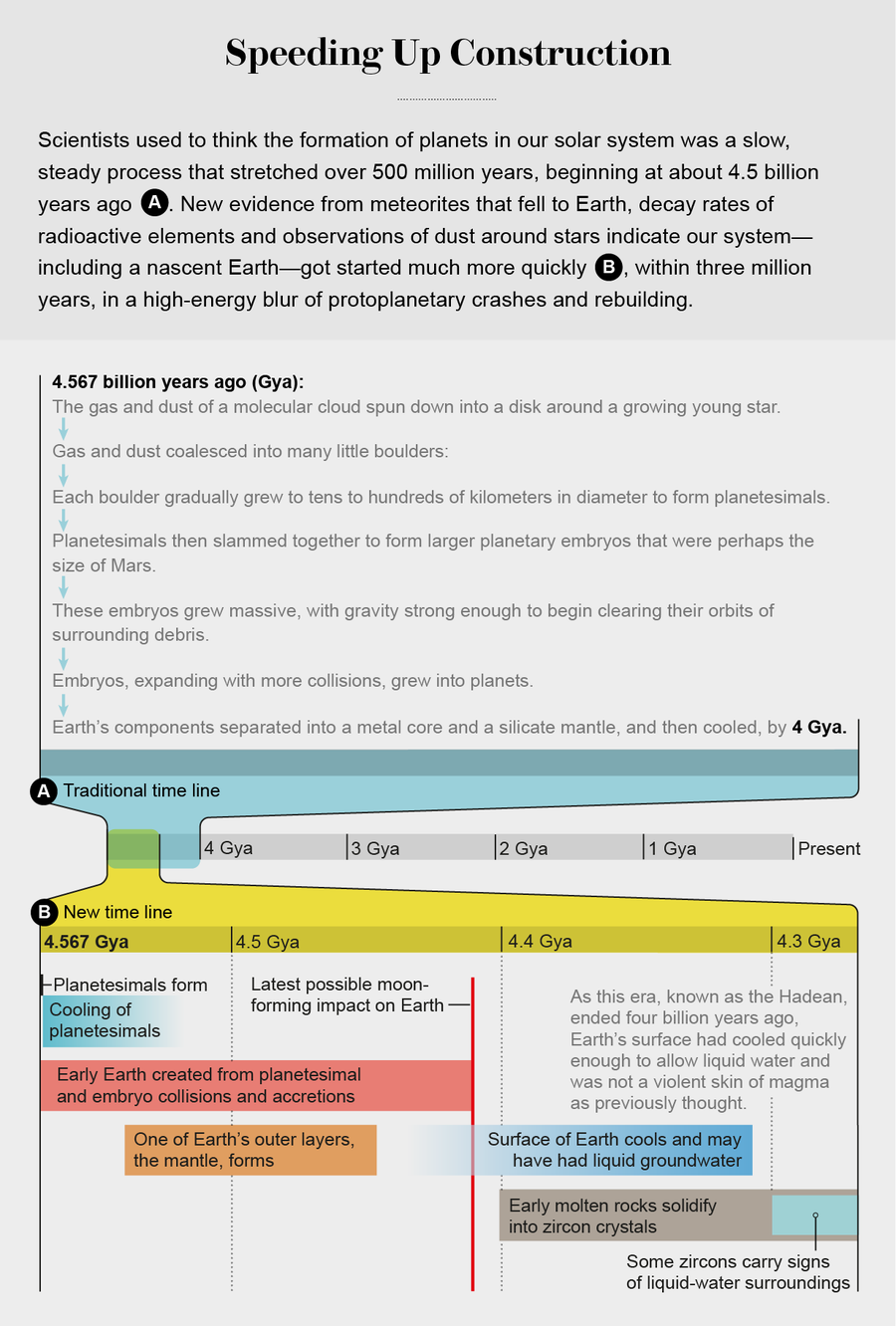
We were not the first to have thought of partial differentiation. Geologist John Wood drew a similar structure by hand in his Ph.D. thesis at M.I.T. in 1958—but no one had ever been heretical enough to say that the poster child for primitive unmelted meteorites, Allende, could have come into existence in this way or that the process was common, indeed formative, at the beginning of our solar system.
Now it does appear common. Researchers have found that at least four other meteorite parent bodies hosted magnetic core dynamos. At the same time, other possible sources of the magnetization have been ruled out: Allende and its fellows were not magnetized by a field produced in the sun, or by the dusty disk itself, or by transient plumes around impacts. If the earliest solar system was truly populated by hundreds or even thousands of differentiated planetesimals, zipping around and generating intense heat and magnetic dynamos like tiny Earths, it implied the entire infant system contained a great deal more heat than geologists had ever thought.
A Crowded Field
Other ideas have also weakened the traditional notion of linear planet growth from small to large. For years, for the sake of simplicity and numerical tractability, all simulations of planet formation assumed that in every collision of planetesimals, all the material from both colliders combined to form a new, bigger body. This merging occurred even as planetesimals were forming from dust. But more recent understanding and approaches to modeling the collision process were pioneered by Erik Asphaug of the University of Arizona. Some collisions are constructive, Asphaug found, and produce larger bodies. But other crashes can be destructive, with the collider stripping material from the target body and continuing on its way to do more damage elsewhere.
Only at about 10 million years of age did bodies really get bigger and stay big. What gave them enough stability to survive? Again, the answer seems to be size. As planetesimals collided and formed larger planetary embryos, their mass and therefore their gravity became larger. The gravity was great enough that whenever their orbits brought them near another object, that object was either drawn in and accreted through the pull of gravity or flung away as its orbit was changed. Thus, these growing planets began to clear out their orbits, which is one of the criteria for being properly called a planet. Smaller bodies had fewer and fewer refuges where their orbits could remain stable and unperturbed by growing planets; the asteroid belt became one of the safe havens left to them.
Mission to a Metal World
Weiss, Asphaug, I and others would like to learn how our own planet's structure and composition came into being out of this energetic, often chaotic environment. But we cannot, despite the science fiction of popular movies or Jules Verne novels, get a good look at Earth's core. It is too deep underground and has pressures too high to sample directly.
But perhaps one particular asteroid, a remnant of an ancient planetesimal, might be a decent stand-in. About 12 years ago several of my colleagues and I began to design a space mission to explore this possibility. We gathered in the Left Field mission-formulation room at NASA's Jet Propulsion Laboratory. This room is designed for creativity. It has shelves full of drawing and building materials such as cardboard, wheels, wire, Legos, paper, markers and foam. It is a good room to think about something completely new, and that is what we were trying to do. We wanted to explore a place that could prove or overturn hypotheses. The best destination, we had decided, was a world made out of metal: the metal asteroid Psyche.
There is nothing quite like this place—at least nothing close enough to reach in a reasonable amount of time. Psyche is one of the largest asteroids, about 200 kilometers in diameter, and is located between Mars and Jupiter. All the physical measurements we have—from radio telescopes bouncing waves off the body—indicate that it is composed of significant amounts of iron and nickel. Psyche looks like it is a stripped-naked planetesimal core, a last remnant of the hit-and-run collisions that disrupted bodies in the early solar system. The orientation of particles in Psyche, like tiny magnetic compass needles, might tell us whether it had a magnetic dynamo. There might also be some remnants of its rocky exterior that tell us what the deep mantle of planetesimals looked like. If there were surface impacts on the naked metal, the splashes might have produced sharp metal cliffs that froze before they could fall back to the surface.
Each of us in the mission room brought a particular set of skills to the table: Weiss, his specialty of measuring magnetic fields in meteorites; William F. Bottke, the dynamics of orbiting bodies; Asphaug, the effects of collisions; Bruce Bills, calculating the gravity field of a body; Daniel Wenkert, managing data and operations. Damon Landau calculated trajectories; he is an interplanetary travel agent. John Brophy organized our deliberations, and I brought my knowledge of compositions, melting, solidification and differentiation processes.
The energy in the room rose to a heady peak. There was no e-mail checking, and there were no conversational pauses. We were united in our excitement over true exploration: humankind has never visited a metal body, and we do not even know what it will look like.
Since then, those days of playing with foam and Legos have given way to an organized push by a team of about 300 people, with more than 1,500 participating in the mission over the years. We proposed a spacecraft about the size of a singles tennis court, powered by solar cells and ion thrusters and carrying a magnetometer to detect magnetic fields, a gamma-ray spectrometer to identify elements and two cameras. In January 2017 we received the good news that NASA had selected the Psyche mission for flight. We will launch the spacecraft in October 2023, and we project that it will arrive at this strange remnant of planet building in 2029. The world of metal awaits.