The Large Hadron Collider (LHC) is the largest particle accelerator in the world. But, after 10 years of operation, it’s time to think about the next steps. With one approved upgrade—the High-Luminosity LHC—and design studies for possible future colliders on the table, intense efforts are being directed to the development of new technologies.
In September 2008, the champagne corks popped in the CERN control room as physicists and engineers celebrated the first beam in the Large Hadron Collider (LHC). It was the beginning of a decade of exciting science, the discovery of the Higgs boson being the highlight. The LHC confirmed many predictions of the standard model of particle physics, but it also raised unsettling questions—such as why the Higgs boson is so light, and why there is no sign of supersymmetry. Ten years on, physicists feel increasing pressure to find answers to these questions. A next-generation particle collider could be what’s needed to reveal physics beyond the standard model.
CERN is on the case. One upgrade is already underway and will be operational in 2026: the High-Luminosity LHC (HL-LHC) will be housed in the LHC tunnel, but with innovative magnets and radiofrequency (RF) cavities that will substantially increase the luminosity (that is, the statistics of the measurements). The energy will be the same as that of the LHC (14 tera electron volts [TeV]), and the plan is to use the HL-LHC to extend the operational lifetime of the LHC until 2040.
On supporting science journalism
If you're enjoying this article, consider supporting our award-winning journalism by subscribing. By purchasing a subscription you are helping to ensure the future of impactful stories about the discoveries and ideas shaping our world today.
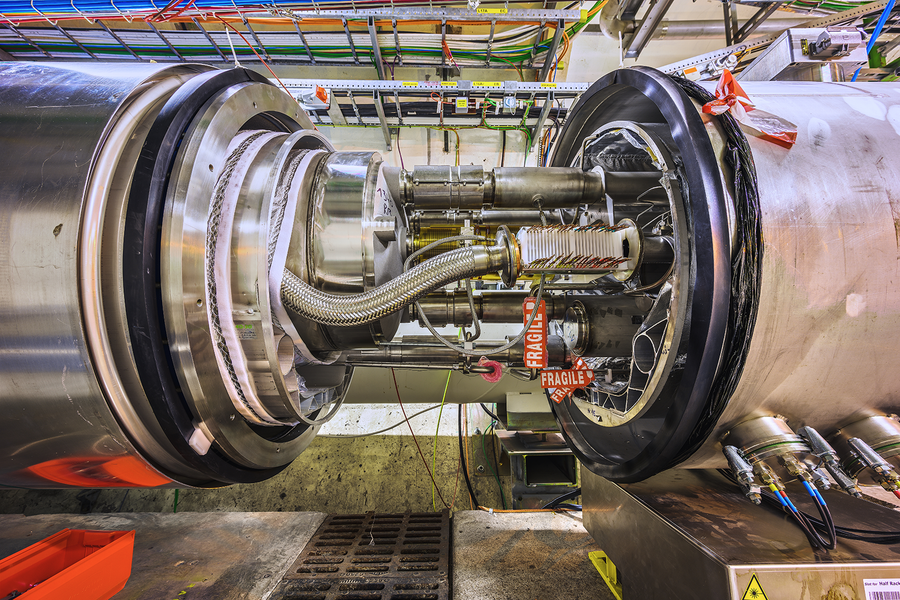
Interconnection between two magnets in the LHC tunnel. Credit: Maximillien Brice and Julien Marius Ordan
But the community is already looking at the future of collider physics beyond 2040, and three proposals for building circular colliders at CERN are on the table. There are two options for a future circular collider (FCC) that would fit in a new 100-km-long tunnel: a lepton-lepton collider or a hadron-hadron collider, with an energy of 100 TeV. Alternatively, on a smaller scale, a high-energy LHC could run in the existing LHC tunnel but use new magnets to reach an energy of 27 TeV. “It could be a long journey if things go well—a journey of several generations,” comments Günther Dissertori, chair of the FCC International Advisory Board and professor of physics at ETH.
A new big circular collider is the preferred option of many at CERN. The lepton and hadron colliders could be implemented one after the other, as it happened for CERN’s large electron-positron collider (LEP) from 1989 to 2000 and then the LHC from 2008. The lepton collider would run for 15 years, performing precision measurements of known particles and possibly measuring deviations from the predictions of the standard model; over this period of time, the technology for the magnets needed for the hadron collider would mature, and the hadron machine could then be used to look for new particles at much higher energies than currently available. “This package is what makes the overall project so interesting; it is the combination that makes the physics,” adds Dissertori.
However, a lot of technological developments are needed, in particular for the RF cavities, which accelerate the particles, and for the magnets, which bend and focus the particle beam. Both will need to be made of high-performance superconducting materials: the cavities to withstand the very high electric fields that will be generated at their interior and the magnets to sustain electrical currents intense enough to generate the desired high magnetic fields, which determine the maximum energy of the beam.
The approved upgrade: HL-LHC
By “luminosity” particle physicists mean the number of collisions that can be produced in a detector per square centimeter per second. More collisions mean better statistics and thus a better chance to study very rare processes. The HL-LHC will have a luminosity 10 times higher than that of the LHC, so the hope is to observe subtle deviations from the predictions of the standard model.
At the heart of the high-luminosity upgrade are magnets made of Nb3Sn—a superconducting material never used before in accelerators. These magnets can reach magnetic fields of up to 12 tesla (the LHC uses Nb-Ti magnets producing fields of 8 tesla). Different designs for the magnets are being tested at CERN and in collaborating laboratories in the U.S., and after two years of work, the performance is approaching the desired specifications. The HL-LHC Nb3Sn magnets will be cooled by 1.9 kelvin superfluid helium but could also operate at 4.2 kelvin, whereas the Nb-Ti magnets of the LHC need to be cooled to 1.9 kelvin. It might seem like a small difference, but the energy savings would be substantial.
Nb3Sn is the superconductor of choice for all future high-field magnets at CERN, but is not straightforward to use. In its superconducting form, Nb3Sn is as brittle as glass and thus cannot withstand a cabling process. Therefore, nonsuperconducting wires composed of Nb and Sn, embedded in a copper matrix, must first be assembled into cables and then heat-treated for several days to react into the superconducting Nb3Sn phase. For HL-LHC, all the cables will be manufactured at CERN or in collaborating labs in the U.S.
.png?w=900)
LHC tunnel during a shutdown period. Credit: Maximillien Brice and Julien Marius Ordan
The other superconducting material that will have an important role in the HL-LHC is MgB2, which has a critical temperature of 39 kelvin and therefore can be cooled with gaseous He. It will be used in the power lines transporting current from the power converters to the accelerator magnets. As a spinoff, researchers at CERN are currently working with companies to develop power lines that will reduce inefficiencies in power distribution.
Another key component that had to be developed for HL-LHC are new RF cavities, called crab cavities, that are made of bulk Nb. They are shaped in a special geometry that tilts the beams to maximize the overlap at the collision point, boosting the number of collisions. The crab cavities are currently being tested in the Super Proton Synchrotron (SPS), one of the older particle accelerators at CERN. Finally, the collimators, which absorb particles that stray from the beam, had to be improved for the HL-LHC to cope with the higher number of particles.
Beyond its scientific mission, the HL-LHC also functions as a validator of new technologies that would be used in the FCC, such as the Nb3Sn magnets. “We need to test the new material, otherwise, who would pay for a FCC that is based on an untested technology?” comments Lucio Rossi, leader of the HL-LHC project.
The proposed upgrade: FCC
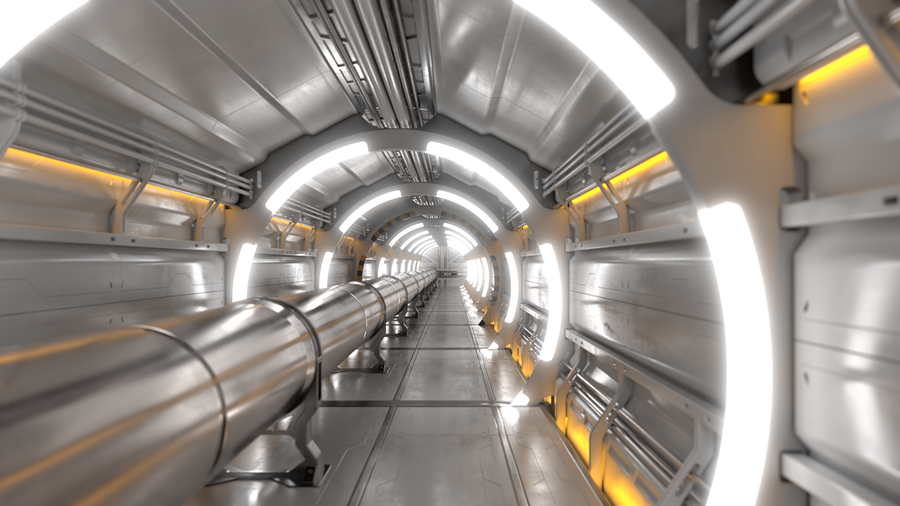
Artistic 3-D view of the proposed new 100-km tunnel that could host different collider. Credit: Panagiotis Charitos
If approved, the FCC will work at the energy frontier of particle accelerators and set the stage for the future of high-energy physics. More than 135 international institutions collaborate on the project. The building works would start in 2028 and be complete in 2040.
The FCC will comprise a 100-kilometer circular accelerator, 16 tesla magnets and next-generation superconducting RF cavities. The ring will be linked to the current accelerators, which will be used as injector machines—indeed, at CERN, every accelerator of the past feeds into the new ones.
A lepton collider could provide ultraprecise measurements of known particles of the standard model to determine their exact parameters. It would collide particles at energies ranging from 90 giga electron volts (GeV) to 365 GeV. These are low compared with the collision energies reached at the LHC, but are relevant for studying the W and Z bosons, Higgs boson and top quarks (which have masses of 80 GeV, 91 GeV, 125 GeV and 173 GeV, respectively). A lepton collider would also work as a Higgs factory, producing billions of Higgs bosons and allowing the detailed investigation of their coupling and interactions.
(1).png?w=900)
Preliminary design of a future detector for a precision-frontier lepton circular collider (FCC-ee). Credit: Panagiotis Charitos
A hadron collider could produce particles with much higher mass (up to 20-30 TeV) that are beyond the energy limits of the LHC. Completely new energy scales would be probed. However, substantial technological developments are needed before the FCC can become reality. Major research and development programs are focused on high-field superconducting magnets and superconducting RF cavities, and, not to forget, a 100-kilometer-long circular tunnel will need to be built. For this, new civil engineering software has been developed to optimize the tunneling in the mountainous region between France and Switzerland. The tunnel would be the longest in the world (the Gotthard tunnel, currently the longest, is 57 kilometers long), and tunneling and infrastructure costs would account for about 30 percent of the FCC budget.
Magnets—it’s all about superconductivity
Although Nb3Sn magnets will be used in the HL-LHC, further improvements are needed to reach the 16-tesla fields that are required for the FCC. Therefore, a major research program at CERN focuses on different options to increase the performance of Nb3Sn magnets, for example, by introducing new production processes and by optimizing the critical current density of Nb3Sn through grain refinement and artificial pinning. “The magnets for future higher energy accelerators require fundamental research on superconductors to achieve the targets in performance and cost,” says Amalia Ballarino, from CERN.
A variety of other superconducting materials are also being developed and tested in labs all over the world, but can often be made only in the form of thin films and are difficult to produce on a large scale. The possibility of using high-temperature superconductors is also being discussed, but these materials are not yet mature enough (even though the design of a proposed big circular collider in China, which would also have a diameter of 100-kilometer, includes high-temperature superconductors for the magnets).
Once the magnets are ready, the interconnections between the different elements in the accelerator also need to be optimized. Therefore, it is important that scientists and engineers collaborate from the beginning. “It is not rocket science, but it is very complex,” says Rossi. “Getting older, I realized the importance of interfaces: if the welding does not work, nothing works.”
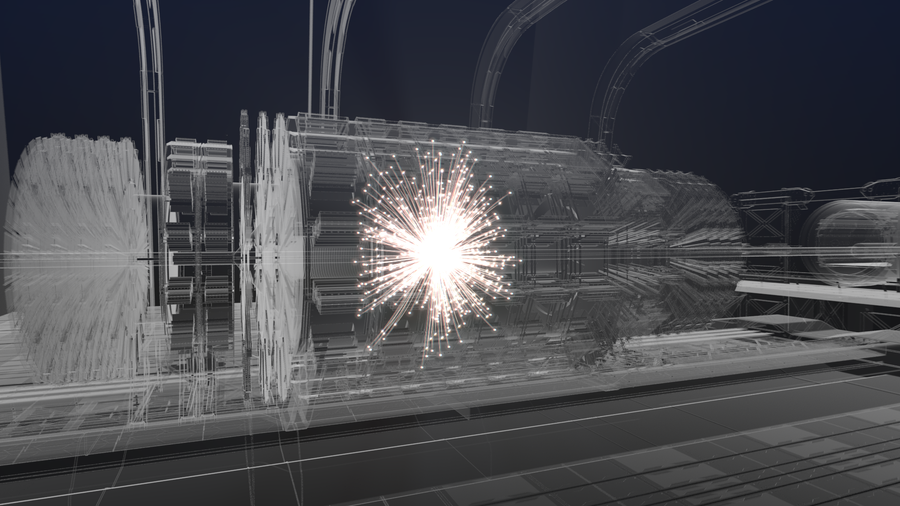
Artistic impression of a collision event. Credit: Panagiotis Charitos
RF cavities—material challenges ahead
In the FCC, energy losses caused by synchrotron radiation will be as high as 100 megawatts. They will need to be compensated by superconducting RF cavities, which will need to accelerate the beam very efficiently; thus, a massive step forward is required in RF cavity research.
The electromagnetic field in the RF cavities oscillates at a specific frequency, providing the electromagnetic field needed to accelerate the particles. The shape and size of the cavities determine the resonance frequency. Various cavity architectures and material processing approaches are currently being tested at CERN. Pure Nb is the superconductor of choice here—there are not many other superconducting materials compatible with RF cavities. In addition, experimental prototype cavities with a Nb3Sn coating have been produced; however, the brittleness of Nb3Sn poses an even greater challenge when it comes to RF cavities, because to make the cavities resonant at a specific frequency (tuning), they have to be slightly deformed. Researchers at CERN are currently also looking at the possibility of coating copper cavities internally with Nb3Sn, using an intermediate tantalum layer to avoid diffusion of copper into the superconducting layer. They are also testing alternative tuning methods to avoid cracking of Nb3Sn.
The future
The LHC is a marvel of engineering. Building an even bigger collider seems unthinkable but, in the words of Captain Jean Luc Picard, ‘‘things are only impossible until they’re not.’’ And it’s not only CERN that is dreaming big: various options for future colliders are being discussed, including a linear collider in Japan and a circular collider in China. But no matter which future collider becomes a reality, beyond their potential for scientific discovery, these big machines will undoubtedly have a huge impact on the progress of material science and engineering.
This article is reproduced with permission and was first published on January 8, 2019.