More than a billion years ago two black holes in the distant universe spiraled around each other in a deathly dance until they merged. This spiraling collision was so violent that it shook the fabric of spacetime, sending perturbations— gravitational waves—rippling outward through the cosmos at the speed of light. In September 2015, after traveling more than a billion light-years, those ripples washed over our planet, registering as a “chirp” in the sensors of the Advanced Laser Interferometer Gravitational-wave Observatory (LIGO).
This was the first direct detection of gravitational waves, and the observation confirmed Albert Einstein’s century-old prediction of their existence. Yet the chirp revealed that each of the merger’s progenitor black holes was 30 times heavier than the sun. That is, their masses were two to three times larger than ordinary black holes born from supernova explosions of massive stars. These black holes were so heavy, it is hard to explain how they formed from stars at all. Furthermore, even if two such black holes did independently form from the deaths of very massive stars, they would have then had to find each other and merge—an event with an exceedingly low probability of occurring within the current age of the universe. It is thus reasonable to suspect that these massive black holes formed via some other, more exotic pathway that might not involve stars at all. Beyond its detection of gravitational waves, it may be that LIGO has unveiled something even more extraordinary: black holes that predate the formation of the stars themselves.
Although such “primordial” black holes have never before been seen, some theoretical models suggest they could have formed in astronomical numbers from the hot, dense plasma that filled the cosmos less than one second after the big bang. This hidden population could solve several outstanding mysteries in modern cosmology. In particular, primordial black holes could constitute some, if not all, of dark matter—the invisible 85 percent of the matter in the universe that acts as gravitational glue to hold galaxies and galaxy clusters together. Further studies with LIGO and other facilities will soon test these ideas, potentially unleashing a new revolution in our understanding of the cosmos.
On supporting science journalism
If you're enjoying this article, consider supporting our award-winning journalism by subscribing. By purchasing a subscription you are helping to ensure the future of impactful stories about the discoveries and ideas shaping our world today.
The Fall of MACHOs, the Rise of WIMPs
Black holes would initially seem to be ideal candidates for dark matter because they emit no light. Indeed, along with other dark objects such as planets and brown dwarfs, they make up one long-proposed solution to the dark matter problem: MACHOs, short for “massive compact halo objects.” Found both in spherical halos surrounding each galaxy and near each galaxy’s luminous center, MACHOs would create the gravitational pull responsible for the otherwise anomalous motions of stars and gas that astronomers observe in the outskirts of galaxies. Simply put, galaxies seem to be rotating too fast to be held together by the visible mass in stars that we observe. Dark matter provides the extra pull to prevent spinning galaxies from flinging off their stars.
If MACHOs make up most of the universe’s dark matter, they must also account for other observations. Whatever dark matter is, it shapes the universe’s largest structures, determining the origin and growth of galaxies, as well as that of galaxy clusters and superclusters. These objects coalesce from the gravitational collapse of clumps of gas inside dark matter halos. Cosmologists have precisely mapped the spatial distribution of these clumps through deep and wide galaxy surveys and correlated them with tiny temperature fluctuations present in the cosmic microwave background (CMB), the big bang’s all-sky afterglow. The diffuse mass of dark matter in large galaxies and clusters also bends space to distort the light from far-distant background objects—a phenomenon known as gravitational lensing.
The MACHO hypothesis, however, fell from favor a decade ago, when MACHOs did not turn up in tentative, indirect searches for their existence. Most notably, astronomers looked for them via microlensing, a variety of gravitational lensing in which a black hole, a brown dwarf or even a planet passes in front of a background star and temporarily magnifies the star’s light. Several multiyear microlensing surveys of millions of stars in the Large and Small Magellanic Clouds, the main satellite galaxies of the Milky Way, found no evidence that MACHOs made up the entirety of our galactic halo. These results were conclusive enough to rule out MACHOs up to around 10 solar masses as the primary constituent of dark matter. As these surveys took place, theorists built the case for an alternative hypothesis—WIMPs, or weakly interacting massive particles.
WIMPs are predicted by certain extensions of the Standard Model of particle physics, but they remain at least as elusive as MACHOs. To date, no evidence of their existence has been found despite decades of searches using particle accelerators, underground detectors and space telescopes. As null results piled up in the search for WIMPs, some researchers began reconsidering the MACHO hypothesis, focusing particularly on primordial black holes. But what process could have seeded these strange objects throughout the observable universe, and how could they have eluded detection for so long?
Black Holes from the Big Bang
Astronomer Bernard Carr and the late physicist Stephen Hawking proposed the idea of primordial black holes in the 1970s. They considered black holes with masses smaller than that of a mountain. Such minuscule black holes would have already evaporated and vanished within the age of our nearly 14-billion-year-old universe, via a quantum-mechanical process discovered by Hawking and appropriately called Hawking radiation. But they also investigated the possibility that more massive, nonevaporating black holes could constitute the missing matter in galaxy clusters. The possibility that massive primordial black holes could actually be most or even all of the dark matter hinges on an idea known as cosmic inflation, first proposed by physicist Alan Guth in the early 1980s.
Inflation is a hypothetical phase of prodigious expansion immediately after the big bang. In 10–35 second, two points separated by less than an atomic radius would have become separated by four light-years, a distance comparable to that of the closest stars. Moreover, during inflation tiny quantum fluctuations are magnified to macroscopic scales by the rapid expansion, seeding the growing universe with underdense and overdense regions of matter and energy from which all cosmic structures subsequently emerge. As bizarre as it may seem, the theory of inflation is strongly supported by observations of such density fluctuations in the CMB.
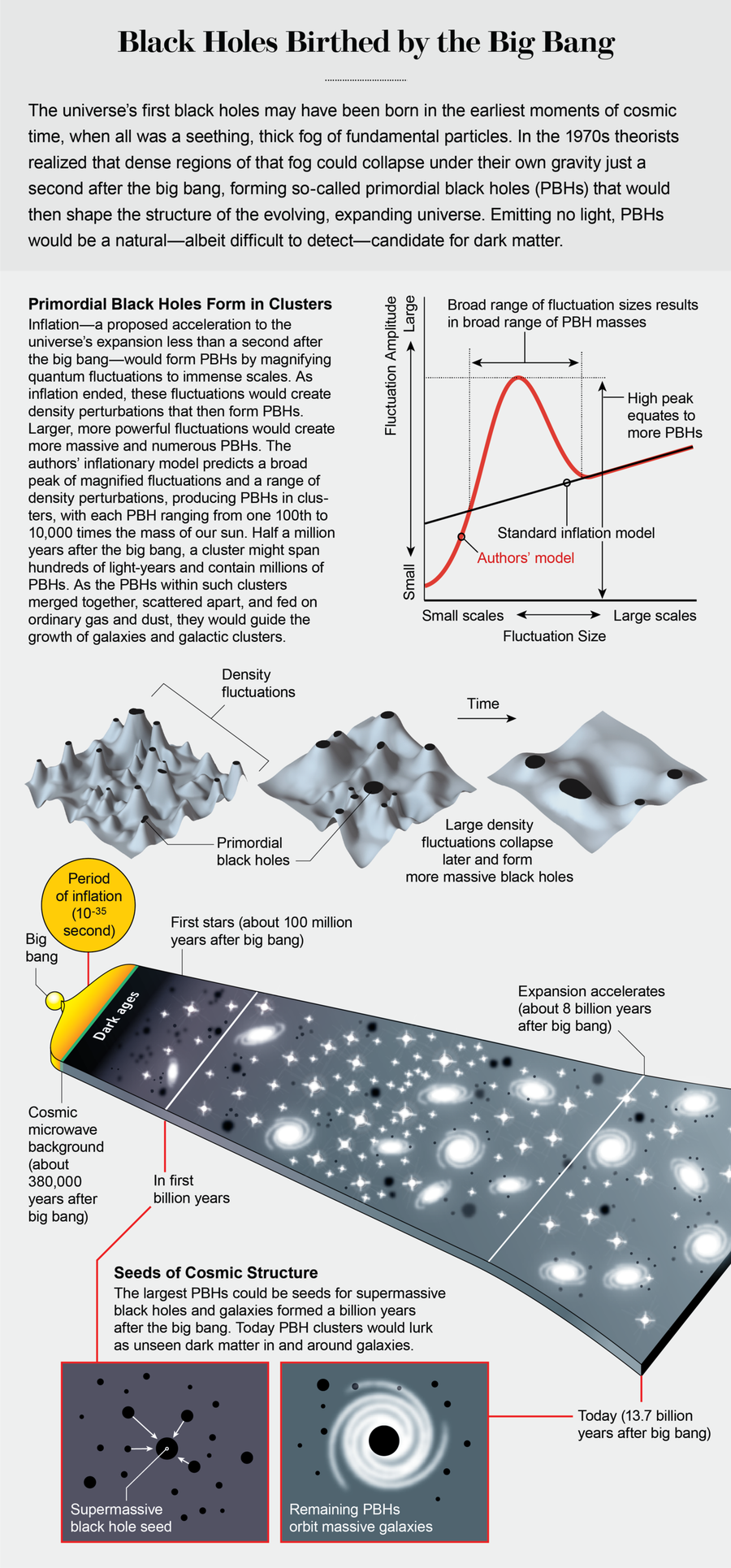
Credit: Jen Christiansen and George Retseck (density-fluctuation panels)
In 1996 one of us (García-Bellido), together with Andrei Linde of Stanford University and David Wands of the University of Portsmouth in England, discovered a way for inflation to form sharp peaks in the spectrum of density fluctuations in the early universe [see box on opposite page]. That is, we showed how quantum fluctuations enormously magnified by inflation would naturally produce particularly dense regions that would collapse to form a population of black holes less than one second after inflation ends. Such black holes would then behave as dark matter and would dominate the matter content of the present-day universe. This model generated a population of black holes with the same mass, determined by the amount of energy within the collapsing region. Many other groups then started exploring these ideas within different models of inflation.
In 2015 the two of us (Clesse and García-Bellido) proposed a scenario, similar to that of 1996, in which these primordial fluctuations exhibit a broad peak in their energy densities and spatial sizes, giving rise to primordial black holes with a wide range of masses. A key consequence of this scenario is the fact that large density fluctuations collapse in close spatial proximity to one another, generating clusters of black holes of different masses—from one 100th to 10,000 times the mass of our sun. Within half a million years of the big bang, each growing, evolving cluster could contain millions of primordial black holes in a volume just hundreds of light-years across.
Such clusters of primordial black holes would be sufficiently dense to explain LIGO’s mysterious black hole mergers, which one would not otherwise expect to occur with regularity. From time to time, the trajectories of two primordial black holes within a cluster can cross, so that both objects become gravitationally bound to each other. They would then spiral closer together for up to millions of years, radiating gravitational waves until they merged. In January 2015 we actually predicted that LIGO would detect gravitational waves from such massive mergers—waves identical to those LIGO then detected later that year. Our estimates for the rate of merger events within primordial black hole clusters fit perfectly within the limits set by LIGO. If LIGO and other similar facilities detect many more mergers, it may be possible to determine the range of masses and spins for all the progenitor black holes. Such a statistical analysis of black hole mergers would provide crucial information for testing their potentially primordial origins.
A key aspect of this scenario is that it evades the constraints on MACHOs previously set by gravitational microlensing experiments—constraints that ruled out black holes of up to about 10 solar masses as the main constituent of dark matter. If primordial black holes exist and possess a wide range of masses, only a small fraction will be visible to these microlensing experiments, with the bulk remaining invisible. And if primordial black holes are grouped in clusters, this arrangement suggests a probability of less than one in 1,000 that a cluster would happen to be along the line of sight of the stars in the nearby satellite galaxies monitored for microlensing events.
To avoid this effect, one could search for microlensing events elsewhere in the sky, looking for the magnified light from stars in the Milky Way’s neighboring Andromeda galaxy or even from quasars in far-distant galaxies. In this way, one could probe a much larger volume of galactic halos for signs of MACHOs—that is, for primordial black holes. Recent observations suggest that whereas MACHOs of up to 10 solar masses may not make up the entirety of an average galaxy’s halo, MACHOs between one tenth and a few solar masses could easily account for about 20 percent of the mass in a typical galactic halo. This value is consistent with our broad-mass primordial black hole scenario.
Simply put, we cannot yet rule out the possibility that dark matter is mostly made up of primordial black holes. Indeed, this proposed scenario could help us decipher several other cosmic mysteries related to dark matter and galaxy formation.
Many Problems, One Solution
Clusters of primordial black holes could clear up the so-called missing-satellite problem—the apparent lack of “dwarf” satellite galaxies that should form around massive galaxies such as our Milky Way. Current simulations modeling the cosmic distribution of dark matter accurately replicate the universe’s observed large-scale structure, in which halos of dark matter pull galaxy clusters into giant filaments and sheets surrounding great voids of lower density. On smaller scales, however, these simulations predict the existence of numerous subhalos of dark matter orbiting around massive galaxies. Each of these subhalos should host a dwarf galaxy, and hundreds should surround the Milky Way. Yet astronomers have found far fewer dwarf galaxies than predicted.
Many potential explanations for the missing-satellite problem exist, mainly the notion that simulations fail to fully account for the influence of ordinary matter (hydrogen and helium in stars) on the formation and behavior of the predicted dwarf galaxies. Our scenario suggests that if clustered primordial black holes made up most dark matter, they would dominate the subhalos surrounding the Milky Way, absorbing a fraction of ordinary matter and reducing the rate of star formation in the subhalos. Moreover, even if these subhalos vigorously formed stars, these stars could easily be ejected by close encounters with massive primordial black holes. Both effects would greatly reduce the brightness of the satellites, making them very hard to detect without wide-field cameras of exquisite sensitivity. Fortunately, such cameras now exist, and astronomers have already used them to discover dozens of ultrafaint dwarf galaxies surrounding the Milky Way. These objects appear to host up to hundreds of times more dark matter than luminous stars, and our model predicts that thousands more should orbit our galaxy.
Simulations also predict a population of galaxies intermediate in size between dwarf galaxies and massive galaxies. Such objects are said to be too big to fail because they would be sufficiently large to readily form stars and be easily seen. Still, they have not turned up in astronomers’ searches of the Milky Way’s vicinity. This too-big-to-fail problem has a solution similar to that of the missing-satellite problem: massive primordial black holes in the cores of intermediate-sized galaxies could eject stars and star-forming gas from these objects, rendering them effectively invisible to most surveys.
Primordial black holes could also resolve the origin of supermassive black holes (SMBHs). These monsters weigh from millions to billions of solar masses and are observed at the centers of quasars and massive galaxies very early in the universe’s history. Yet if these SMBHs formed and grew from the gravitational collapse of the universe’s first stars, they should not have acquired such gigantic masses in such a relatively short time—less than a billion years after the big bang.
In our scenario, although most primordial black holes have just tens of solar masses, a very small fraction will be far heavier, ranging from hundreds to tens of thousands of solar masses. Born less than a second after the big bang, these monstrous objects would then act as giant seeds for the formation of the first galaxies and quasars, which would rapidly develop SMBHs at their centers. Such seeds could also account for the existence of intermediate-mass black holes possessing 1,000 to a million solar masses observed orbiting SMBHs and at the centers of globular clusters of stars. In short, primordial black holes may be the missing link between conventional stellar-mass black holes and SMBHs. The observational case for this scenario is building rapidly: recent detections of unexpectedly abundant x-ray sources in the early universe are most easily explained by large numbers of primordial black holes producing x-rays as they gorge on gas less than one billion years after the big bang.
Seeing in the Dark
Even though massive primordial black holes could solve the mystery of dark matter, as well as many other long-standing problems of cosmology, the game is not yet over. Other models and explanations are still possible, and future observations should allow us to distinguish among the alternatives. Indeed, there already exist seven kinds of observations to test the primordial black hole scenario [see box on opposite page]. They include the detection of ultrafaint dwarf galaxies, the influence of massive primordial black holes on the positions of stars in the Milky Way, the mapping of neutral hydrogen during the first epoch of star formation and future observations of distortions in the cosmic microwave background.
Beyond these experiments, we also now possess a completely new tool to unravel the mysteries of the universe in the form of Advanced LIGO and other gravitational-wave detectors. If indeed LIGO has detected merging members of a hidden population of massive primordial black holes, we should expect many more to be detected in coming years. Before the end of the third run, scientists from the Virgo collaboration in Italy and the Advanced LIGO had presented 50 confirmed detections of gravitational waves emitted during the merging of black hole binaries, and many new detections are expected in future runs. These detections suggest that binary black holes are much more frequent than expected and that they are broadly distributed in mass, in agreement with our scenario of massive primordial black holes.
Taken together these new experiments and observations could confirm the existence of primordial black holes and their possible linkage to the universe’s missing matter. Soon we may no longer be in the dark about dark matter.